High hydrogen evolution activities of dual-metal atoms incorporated N-doped graphenes achieved by coordination regulation
Abstract
Electrolysis of water to produce hydrogen (H) can solve the current energy crisis and environmental problems. However, efficient hydrogen evolution reaction (HER) catalysts are still limited to a few noble metals, thus prohibiting their broad applications. Herein, first-principles calculations were carried out to investigate the theoretical HER performances of a series of N-doped graphenes containing inexpensive single- and dual-metal atoms. Among them, MN4-gra (M = Fe, Co, Ni), homonuclear MMN6-gra, and heteronuclear M1M2N6-gra mostly exhibit low HER activities due to the weak H adsorption, and only CoN4-gra, NiNiN6-gra, and CoNiN6-gra show better ΔG*H values of 0.19, 0.15 and 0.27 eV, respectively. In contrast, low-coordinated MMN5-gra and M1M2N5-gra both have rather high HER activities. In particular, the ΔG*H values of FeNiN5-gra and CoNiN5-gra are as low as
Keywords
INTRODUCTION
The massive consumption of fossil resources and the increasingly serious environmental problems prompt people to search for suitable alternative energy sources and develop efficient energy conversion technologies. However, the lack of cheap and high-efficiency catalysts largely restricts the overall efficiency of energy conversion devices and is the main bottleneck for realizing the transformation of energy supply forms. As important chemical reactions, hydrogen evolution reactions (HER) and oxygen evolution reactions (OER) form the whole reaction of water electrolysis to produce hydrogen (H)[1-4]. The generated hydrogen can then be used in fuel cells as a carrier of clean energy due to its high mass energy density[5-7]. Unfortunately, the current utilization of hydrogen energy is still in its infancy due to the lack of cheap and efficient HER catalysts. As an optimal HER catalyst, platinum (Pt) requires only negligible overpotentials in acidic solutions to achieve high reaction rates[8-11]. However, the scarcity limits its large-scale application. Although many HER catalysts such as transition-metal phosphides[12-14], carbides[15-17] and sulfides[18,19] have been developed, the excellent performance as that of Pt/C is still hard to achieve.
In recent years, single-atom catalysts (SACs), exemplified by the metal atoms anchored on nitrogen (N)-doped graphenes (M-N-C), exhibit excellent catalytic activities in many important electrocatalytic processes[20-22]. The unique structure of SACs achieves nearly 100% atomic utilization and excellent catalytic performance[23-25]. Thus, they become promising catalyst materials for HER[26-32]. For example, Lu et al. prepared a Ru and N co-doped carbon material (RuCxNy) as an efficient HER catalyst[33]. When the overpotential is only -12 mV, the current density can reach 10 mA·cm-2, and the catalytic performance is significantly better than that of commercial Pt-based catalysts. Its excellent catalytic activity is mainly attributed to the embedded Ru atoms in the carbon matrix. First-principles calculations indicate that
Compared with SACs, dual-atom catalysts (DACs) not only maintain the high atomic utilization and good selectivity and stability but also have higher metal loading and more complex and flexible active sites. The possible synergistic effect, orbital coupling, and electron redistribution between adjacent metal centers and their functionality complementarity provide more opportunities for better catalytic performance[34-36]. Thus, they have attracted more interest than SACs. For example, Zheng et al. constructed a series of N-doped porous graphene (NPG)-based diatomic catalysts MM’-NPG and studied their HER activity[37]. Due to the dual active centers and controllable electronic structure, FeV-NPG and NiV-NPG could replace the noble metal HER catalysts under alkaline conditions. Zhou et al. synthesized the Rh-Fe dual atoms-embedded N-doped carbon hollow spheres, which exhibit a low overpotential of 36 mV for HER due to the electron redistribution promoted by Fe on the active Rh site[38]. Zhao et al. reported that the presence of C1-Pt-Ru-N2 structures in Pt1Ru1/NMHCS-A (activated N-doped mesoporous hollow carbon spheres) can greatly accelerate the H2 generation with a rather low overpotential of 22 mV to achieve a current density of
To obtain potential HER catalysts, we herein constructed a series of single-/dual-metal atom-incorporated N-doped graphenes with different coordination environments and systematically investigated their HER activities by density functional theory (DFT) calculations. The potential active centers of various catalysts and the adsorption structures of the key *H intermediate were investigated in detail. The HER activity of each catalyst was evaluated by calculating the free energy of H adsorption, and some very promising HER catalysts were then proposed. Finally, in-depth electronic structure analyses revealed the activity origins of the catalysts to provide a theoretical guide for the rational design of related catalytic materials.
COMPUTATIONAL METHODS
DFT calculations considering spin polarization were carried out by using the Vienna ab initio simulation package (VASP)[41]. The Perdew-Burke-Ernzerhof (PBE)[42] functional and the projector augmented wave (PAW) method are used. The dispersion interactions during adsorption were considered by using the popular Grimme’s DFT-D3 correction method[43]. The convergence thresholds are 10-5 eV and 0.02 eV/Å for the energy and force, respectively, and the cutoff energy for the plane wave basis set is 500 eV. A vacuum space of 15 Å was applied along the z-direction to avoid the interactions between slabs. A 2 × 2 × 1 Monkhorst-Pack k-point mesh was used because the cell is as large as 17.22 Å × 17.22 Å × 15.04 Å. All these parameters have been carefully tested in our recent work[44]. All the results were visualized with the aid of the VESTA software[45].
To evaluate the structural stability of metal atoms in the N-doped graphene, the formation energy (taking low-coordinated ones as an example) was calculated as:
or
where EMN3-gra and EN3-gra are the calculated energies of SACs and corresponding N-doped graphene without the metal atom, respectively. Similarly, EMMN5-gra and EN5-gra are the homonuclear DAC and corresponding metal-free structures, respectively. EM denotes the calculated energy of a metal atom in its stable bulk structure. Therefore, a more stable metal site has a more negative Ef value.
The adsorption Gibbs free energies were calculated as:
where the adsorption energies of the first and second H atoms are calculated as ΔE*H1 = E*H1- E* - 1/2EH2 and ΔE*H2 = E*H2- E*H1- 1/2EH2, respectively. E*H1 and ΔE*H2 are the energies of the systems with one and two adsorbed H atoms, respectively. E* is the energy of the catalyst. EH2 is the energy of a free H2 molecule. ΔZPE is the change in zero-point energy upon adsorption, while ΔS represents the change in entropy. They were obtained from the vibrational frequency calculations and standard thermodynamic data, as done in the recent literature[46]. T is the temperature (298.15 K). We assume pH = 0 and applied potential U = 0 V vs. Standard hydrogen electrodes (SHE) in the calculations. The computational hydrogen electrode (CHE) model[47] was used to calculate the Gibbs free energy change, namely assuming the free energy of an isolated H2 molecule is twice that of a proton-electron pair. For simplicity, the hydrogen coverage was not considered, and it is assumed to have a negligible effect as it does on other catalyst surfaces[46].
RESULTS AND DISCUSSION
MN4-gra/MMN6-gra/M1M2N6-gra
We first investigated the nine MN4-gra (M = Fe, Co, Ni), MMN6-gra, and M1M2N6-gra catalysts, and Figure 1 gives their typical structures with each metal surrounded by four N atoms. Our recent study revealed that some MMN6-gra and M1M2N6-gra catalysts exhibit excellent OER activity due to the rich site synergy effects[44]. They are all planar, and their structural stabilities have been confirmed. Herein, we turned to investigate their possible HER activities.
Figure 1. Potential active centers of each type of catalyst. (A) MN4-gra (M = Fe, Co, Ni); (B) MMN6-gra; (C) M1M2N6-gra.
In the acidic solution (pH = 0), the abundant H+ in the electrolyte can be adsorbed on the catalyst under a certain external voltage to form a *H-rich surface, which then reacts to generate hydrogen molecules. Therefore, it is important to study the adsorption behavior of hydrogen on the catalyst surface, with the free energy change (ΔG*H) of the adsorption process being the most critical activity descriptor of a HER catalyst[8]. The ideal catalyst should have a zero ΔG*H value as a result of balanced adsorption and desorption. Figure 1 shows all possible H adsorption sites of these catalyst models. The geometric optimization of the H adsorption configuration on their surfaces was carefully performed to ensure that the most favorable H adsorption structure and adsorption site were found for each of them. Note that besides the metal and carbon sites considered in our recent OER study[44], the N atoms coordinating with metals were also included in this work because they are widely regarded as potential HER active centers[33,37,48]. Supplementary Tables 1-9 summarize the adsorption structures and corresponding ΔG*H values on all these active sites of the above nine catalysts.
First, for the metal sites, the hydrogen atom tends to be vertically adsorbed on most metals except that the one on the Ni site of FeNiN6-gra is slightly close to the Fe site. The adsorption behavior of hydrogen on metal sites is basically consistent with the d-band center theory[49]. Compared with FeN4-gra, the adsorption at Fe sites of FeFeN6-gra, FeCoN6-gra, and FeNiN6-gra is weakened due to the formation of M-M bonds and the lowered metal d-band center (εd, Supplementary Table 10). They, thus, show lower HER catalytic activity (ΔG*H ≥ 0.37 eV) than FeN4-gra (ΔG*H: 0.34 eV). Consistent with the d-band center order of Co atoms (CoN4-gra > CoNiN6-gra > FeCoN6-gra > CoCoN6-gra)[44], their corresponding ΔG*H values are 0.19, 0.62, 0.79, and 0.84 eV, respectively. Among them, CoN4-gra has the best HER catalytic activity with ΔG*H of only 0.19 eV, which is well consistent with its high activity in recent experiments[48]. Interestingly, the hydrogen atom tends to adsorb on the non-metal sites (N or C sites) of NiN4-gra instead of the Ni site. Compared with NiN4-gra, the Ni site on NiNiN6-gra exhibits enhanced H adsorption owing to its upshifted d-band center. Nevertheless, its H adsorption is still weak (ΔG*H: 1.34 eV). The Ni sites of FeNiN6-gra and CoNiN6-gra exhibit weaker H adsorption due to the relatively strong Fe-Ni and Co-Ni interactions and the downshifted d-band center of Ni atoms. Thus, the Ni sites on these catalysts may be unsuitable for HER.
Then, all the non-metal active sites, namely the N atoms coordinating with metals and the C atoms coordinating directly with N atoms, were investigated. Supplementary Tables 1-9 show that besides the vertical adsorption on top of the N atom, the hydrogen atom on the N site may also reside slightly towards the metal site in some cases. The H adsorption strengths of N sites in different coordination environments are quite different. The N sites on MN4-gra have weak H adsorption (ΔG*H ≥ 1.05 eV, Figure 2), implying their low HER activity. For the N sites with two different coordination environments (N1, N2) on
Figure 2. Gibbs free energy of H adsorption (ΔG*H) at different sites on MN4-gra/MMN6-gra/M1M2N6-gra. For the non-metal sites, only the values with the strongest adsorption are shown. Carbon: brown; Iron: golden; Cobalt: dark blue; Nickel: silvery; Nitrogen: light blue.
In summary, the metal sites of the above catalysts mostly exhibit weak adsorptions, and only the Co site of CoN4-gra shows potential high HER activity (ΔG*H: 0.19 eV). For the same reason, all the carbon sites are unsuitable for HER. Interestingly, owing to the dual-atom doping, the N2 sites simultaneously coordinated with the two metal atoms present enhanced H adsorption. In particular, the moderate H adsorptions on these N sites render NiNiN6-gra as a potential HER catalyst with ΔG*H as low as 0.15 eV. The overall weak H adsorptions on the above catalysts [Figure 2] necessitate further tailoring of their structures to achieve better activity.
MN3-gra/MMN5-gra/M1M2N5-gra
Theoretically, the HER performance of the above catalysts could be improved by reducing the number of coordinating N atoms because it will endow the central metal with a stronger coordination ability to enhance its H adsorption. We then constructed a series of SACs and DACs with three N atoms around each metal: MN3-gra, MMN5-gra, and M1M2N5-gra[50,51]. However, the metal atoms in MN3-gra (M = Fe, Co, Ni) SACs all protrude from the catalyst surface upon structural optimization [Supplementary Figure 1]. The formation energies of MN3-gra are also positive, suggesting that they may be unstable and unsuitable for electrocatalytic reactions.
By comparison, the optimized structures of MMN5-gra/M1M2N5-gra DACs are all basically planar [Figure 3], with each metal atom well incorporated in the graphene plane. The metal atom and three nearby N atoms have average distances between 2.03~2.14 Å, all close to the sum of the covalent atomic radii
Figure 3. Optimized structures (two views) and formation energies (Ef) of (A-C) MMN5-gra (M = Fe, Co, Ni) and (D-F) M1M2N5-gra. The first column gives the different active sites considered for each kind of catalyst.
The electronic properties were then investigated by the density of states (DOSs) and electronic band structures. As shown in Supplementary Figure 5, substantial hybridization was observed between the N-2p state and the M-3d state. Compared with MMN6-gra/M1M2N6-gra, the metal d-band centers (εd) in
The changed electronic structure caused by the reduced metal coordination number will inevitably affect the adsorption of *H. Supplementary Tables 13-18 summarize the adsorption structures and corresponding ΔG*H on different active sites of all these low-coordinated DACs. For the metal sites, the H atom prefers to adsorb on the bridge site between two metal atoms of MMN5-gra/M1M2N5-gra as compared to a single metal site on MMN6-gra/M1M2N6-gra. Owing to such a novel dual-atom synergy, the ΔG*H values decrease significantly [Figure 4] and are very close to 0 eV on the metal sites of FeFeN5-gra (-0.16 eV), FeCoN5-gra
Figure 4. Gibbs free energy of H adsorption (ΔG*H) at different sites on MMN5-gra/M1M2N5-gra. For the non-metal sites, only the values with the strongest adsorption are shown.
For the C sites, their overall adsorptions are still weak because their coordination environments in
Dual-H co-adsorption path
When the metal coordination of the catalyst is unsaturated, the HER may also occur along a new reaction pathway[52-57]: after the formation of a usual MH species on the catalyst surface, a second H atom continues to be adsorbed on the same metal site to form a stable HMH intermediate. Thus, with low metal coordination numbers, the possibility for MMN5-gra/M1M2N5-gra to adsorb a second H atom was further investigated. The corresponding optimized structures are summarized in Supplementary Table 19. Indeed, all these catalysts formed stable HMH structures with one H atom on a metal and one H atom between two metals. The corresponding H-H distances are all no more than 1.86 Å. We calculated the adsorption free energy of the second H to evaluate its adsorption strength [Supplementary Table 20]. Most catalysts show favorable adsorption for the second H, especially the Ni site of NiNiN5-gra (ΔG*H2 = -0.09 eV) and the Co site of FeCoN5-gra (ΔG*H2 = -0.11 eV), suggesting that stable HMH structures could form on the surface of these catalysts.
Recently, Liberto et al. found that if the H2 generation involves two reaction intermediates, MH and HMH, an excellent HER catalyst should be thermoneutral for every H adsorption[58,59]. Namely, the ΔG*H values for both adsorption steps (ΔG*H1 and ΔG*H2) should be close to 0 eV. We further evaluated the catalyst activity under this HER mechanism. As shown in Figure 5, FeNiN5-gra may have the best activity under this mechanism via the adsorption at its Ni site. In addition, CoNiN5-gra can achieve rather high catalytic activity either through Co sites (ΔG*H1: -0.06 and ΔG*H2: 0.10 eV) or through Ni sites (ΔG*H1: -0.06 and ΔG*H2: 0.18 eV). The other two promising candidates are FeFeN5-gra and FeCoN5-gra via their Fe and Co sites, respectively.
Figure 5. Adsorption Gibbs free energy of the first (ΔG*H1) and second H (ΔG*H2) in the HMH intermediates on different catalyst sites.
In conclusion, most of the MMN5-gra/M1M2N5-gra exhibited excellent HER activity through the MH intermediate reaction mechanism or through the HMH intermediate mechanism. In particular, the |ΔG*H| values of FeNiN5-gra and CoNiN5-gra under both reaction mechanisms are all no more than 0.1 eV, indicating that they are potential HER catalysts. Supplementary Figure 8 depicts the calculated exchange currents for the above catalyst models based on their adsorption free energies. Among them, CoN4-gra, NiNiN6-gra, FeNiN5-gra, CoNi-N5-gra, FeFeN5-gra, and FeCoN5-gra reside much closer to the volcano peak with high activity.
Origins of HER activity
The above calculation results show that CoN4-gra, NiNiN6-gra, CoNiN6-gra, and MMN5-gra/M1M2N5-gra all have better activities for catalyzing HER. However, the underlying reasons for their enhanced activities may be different.
For CoN4-gra, its excellent HER activity can be attributed to the moderate adsorption of H on Co sites. To understand the huge difference in the H adsorption capacity of different metal sites, the charge transfer between metal atoms as HER active sites and adsorbed hydrogen atoms was further investigated. The charge density difference shows that charge accumulation (yellow area) mainly occurs on the adsorbed H atoms, whereas charge depletion (cyan area) is concentrated around the metal atoms [Supplementary Figure 9]. In addition, the results of Bader charge analysis show that the adsorption free energy of H increases with the decrease of the negative charge it carries; that is, the adsorption gradually weakens. For example, the negative charges carried by H on CoN4-gra and CoCoN6-gra are -0.07 and -0.03 [Figure 6A and B], respectively, and the adsorption free energies of H are correspondingly 0.19 and 0.84 eV.
Figure 6. Charge density difference and Bader charge values during the hydrogen evolution reaction, (A) CoN4-gra and (B) CoCoN6-gra. COHP and DOS between M-H in *H of (C) CoN4-gra and (D) CoCoN6-gra. COHP: Crystal orbital Hamiltonian population; DOS: density of state.
The COHP between the metal atoms and the adsorbed H atoms was further calculated [Supplementary Figure 10]. The activation of H was also quantitatively assessed using the ICOHP. In general, a more negative ICOHP corresponds to a stronger H adsorption. For example, the Co-H interaction in CoN4-gra (ICOHP: -3.28, Figure 6C) is much stronger than that in CoCoN6-gra (ICOHP: -3.08, Figure 6D). A clear linear correlation between the ICOHP of M-H and the free energy of H adsorption for all MN4-gra/
Figure 7. (A) Linear relationship between ICOHP and free energy of H adsorption for MN4-gra/MMN6-gra/M1M2N6-gra; (B) Linear relationship between the d-band centers of the metals and free energy of H adsorption. ICOHP: M-H crystal orbital Hamiltonian population integral.
The enhanced H adsorption capacity of MMN5-gra/M1M2N5-gra is consistent with the reduced coordination number and upshifted d-band center of the central metal [Supplementary Figure 5 and Supplementary Table 10]. Besides, the bridge site between two metals of MMN5-gra/M1M2N5-gra also helps to enhance their capture abilities. Further charge density difference and Bader charge analyses revealed that both metal atoms on MMN5-gra/M1M2N5-gra transfer electrons to the adsorbed H atoms, which results in a significant increase in the amount of charge on H (≤ -0.15, Figure 8) compared to the less negative charge of H on MMN6-gra/M1M2N6-gra (≥ -0.11, Supplementary Figure 9). In addition, according to the COHP analyses [Supplementary Figure 11], the bimetallic atoms on MMN5-gra/M1M2N5-gra simultaneously have bonding interactions with the adsorbed H atoms. This further confirms that the dual atoms in MMN5-gra/M1M2N5-gra both contribute to the H adsorption. Meanwhile, the excellent HER activity of MMN5-gra/M1M2N5-gra to generate H2 through HMH intermediates can also be attributed to the enhancement of H adsorption capacity after reducing the coordination number of metal active centers and the synergistic effect of bimetallic site on H adsorption.
Figure 8. Charge density difference and Bader charge when the hydrogen evolution reaction occurs on the metal site for
Finally, the HER activity of NiNiN6-gra and CoNiN6-gra is mainly due to the optimized electronic structure of the N2 site by doping bimetallic atoms. Specifically, the p-band center (εp)[60] of the N2 site in MMN6-gra and M1M2N6-gra is upshifted relative to that of MN4-gra due to the direct coordination with two metal atoms [Figure 9]. This leads to a significant enhancement of the H adsorption capacity of N2 sites on
CONCLUSIONS
In summary, we investigated the HER activity of a series of SACs/DACs formed by embedding inexpensive Fe/Co/Ni atoms into N-doped graphene by systematic DFT calculations and found a variety of potential HER materials. Besides the CoN4-gra, the ΔG*H values of NiNiN6-gra and CoNiN6-gra are also as low as 0.15 and 0.27 eV, respectively. Reducing the coordination number of the metal in the active center can significantly enhance its H-trapping ability, and FeFeN5-gra, FeCoN5-gra, FeNiN5-gra, and CoNiN5-gra, thus, exhibit excellent HER catalytic activity with |ΔG*H| ≤ 0.18 eV. Importantly, the reduced metal coordination of MMN5-gra/M1M2N5-gra and the synergy between metal atoms allow them to simultaneously adsorb two hydrogen atoms to form a stable HMH intermediate toward the final product H2. Especially for FeNiN5-gra and CoNiN5-gra, both the |ΔG*H1| and |ΔG*H2| are ≤ 0.10 eV. The effective coordination regulation and site synergy revealed by this work provide deep insights into the HER process on diatomic catalysts and could guide the design of low-cost catalysts with activity comparable to noble-metal ones.
DECLARATIONS
Authors’ contributions
Conceptualization, investigation, writing - original draft: Zhang C
Conceptualization, supervision, resources, writing - review and editing: Jin P
Investigation and data analysis and interpretation: Qin S, Gao H
Availability of data and materials
Not applicable.
Financial support and sponsorship
This work was supported by the National Natural Science Foundation of China (No. 22171068) and the Natural Science Foundation of Hebei Province (No. B2022202036).
Conflicts of interest
All authors declared that there are no conflicts of interest.
Ethical approval and consent to participate
Not applicable.
Consent for publication
Not applicable.
Copyright
© The Author(s) 2024.
Supplementary Materials
REFERENCES
1. Li L, Wang P, Shao Q, Huang X. Metallic nanostructures with low dimensionality for electrochemical water splitting. Chem Soc Rev 2020;49:3072-106.
2. Jiao Y, Zheng Y, Jaroniec M, Qiao SZ. Design of electrocatalysts for oxygen- and hydrogen-involving energy conversion reactions. Chem Soc Rev 2015;44:2060-86.
3. Huang J, Jiang Y, An T, Cao M. Increasing the active sites and intrinsic activity of transition metal chalcogenide electrocatalysts for enhanced water splitting. J Mater Chem A 2020;8:25465-98.
4. Kim HJ, Kim HY, Joo J, et al. Recent advances in non-precious group metal-based catalysts for water electrolysis and beyond. J Mater Chem A 2022;10:50-88.
6. Liu M, Zhang R, Chen W. Graphene-supported nanoelectrocatalysts for fuel cells: synthesis, properties, and applications. Chem Rev 2014;114:5117-60.
7. Majlan E, Rohendi D, Daud W, Husaini T, Haque M. Electrode for proton exchange membrane fuel cells: a review. Renew Sust Energ Rev 2018;89:117-34.
8. Nørskov JK, Bligaard T, Logadottir A, et al. Trends in the exchange current for hydrogen evolution. J Electrochem Soc 2005;152:J23.
9. Seh ZW, Kibsgaard J, Dickens CF, Chorkendorff I, Nørskov JK, Jaramillo TF. Combining theory and experiment in electrocatalysis: insights into materials design. Science 2017;355:eaad4998.
10. Xu H, Cheng D, Cao D, Zeng XC. A universal principle for a rational design of single-atom electrocatalysts. Nat Catal 2018;1:339-48.
11. Benck JD, Hellstern TR, Kibsgaard J, Chakthranont P, Jaramillo TF. Catalyzing the hydrogen evolution reaction (HER) with molybdenum sulfide nanomaterials. ACS Catal 2014;4:3957-71.
12. Du H, Kong RM, Guo X, Qu F, Li J. Recent progress in transition metal phosphides with enhanced electrocatalysis for hydrogen evolution. Nanoscale 2018;10:21617-24.
13. Sun J, Ren M, Yu L, et al. Highly efficient hydrogen evolution from a mesoporous hybrid of nickel phosphide nanoparticles anchored on cobalt phosphosulfide/phosphide nanosheet arrays. Small 2019;15:e1804272.
14. Yan Q, Chen X, Wei T, et al. Hierarchical edge-rich nickel phosphide nanosheet arrays as efficient electrocatalysts toward hydrogen evolution in both alkaline and acidic conditions. ACS Sustainable Chem Eng 2019;7:7804-11.
15. Gao Q, Zhang W, Shi Z, Yang L, Tang Y. Structural design and electronic modulation of transition-metal-carbide electrocatalysts toward efficient hydrogen evolution. Adv Mater 2019;31:1802880.
16. Han N, Yang KR, Lu Z, et al. Nitrogen-doped tungsten carbide nanoarray as an efficient bifunctional electrocatalyst for water splitting in acid. Nat Commun 2018;9:924.
17. Zhou S, Zhou G, Jiang S, Fan P, Hou H. Flexible and refractory tantalum carbide-carbon electrospun nanofibers with high modulus and electric conductivity. Mater Lett 2017;200:97-100.
18. Zhang B, Liu J, Wang J, et al. Interface engineering: The Ni(OH)2/MoS2 heterostructure for highly efficient alkaline hydrogen evolution. Nano Energy 2017;37:74-80.
19. Ling Y, Yang Z, Zhang Q, Zhang Y, Cai W, Cheng H. A self-template synthesis of defect-rich WS2 as a highly efficient electrocatalyst for the hydrogen evolution reaction. Chem Commun 2018;54:2631-4.
20. Pan Y, Liu S, Sun K, et al. A bimetallic Zn/Fe polyphthalocyanine-derived single-atom Fe-N4 catalytic site:a superior trifunctional catalyst for overall water splitting and Zn-air batteries. Angew Chem Int Ed Engl 2018;57:8614-8.
21. Cao L, Luo Q, Chen J, et al. Dynamic oxygen adsorption on single-atomic Ruthenium catalyst with high performance for acidic oxygen evolution reaction. Nat Commun 2019;10:4849.
22. Varela AS, Ju W, Strasser P. Molecular nitrogen-carbon catalysts, solid metal organic framework catalysts, and solid metal/nitrogen-doped carbon (MNC) catalysts for the electrochemical CO2 reduction. Adv Energy Mater 2018;8:1703614.
23. Yao Y, Hu S, Chen W, et al. Engineering the electronic structure of single atom Ru sites via compressive strain boosts acidic water oxidation electrocatalysis. Nat Catal 2019;2:304-13.
24. Wang X, Li Z, Qu Y, et al. Review of metal catalysts for oxygen reduction reaction: from nanoscale engineering to atomic design. Chem 2019;5:1486-511.
25. Zhao Y, Zhou H, Chen W, et al. Two-step carbothermal welding to access atomically dispersed Pd1 on three-dimensional zirconia nanonet for direct indole synthesis. J Am Chem Soc 2019;141:10590-4.
26. Lei Y, Wang Y, Liu Y, et al. Designing atomic active centers for hydrogen evolution electrocatalysts. Angew Chem Int Ed Engl 2020;59:20794-812.
27. Wang Y, Su H, He Y, et al. Advanced electrocatalysts with single-metal-atom active sites. Chem Rev 2020;120:12217-314.
28. Zhuo HY, Zhang X, Liang JX, Yu Q, Xiao H, Li J. Theoretical understandings of graphene-based metal single-atom catalysts: stability and catalytic performance. Chem Rev 2020;120:12315-41.
29. Khalid M, Bhardwaj PA, Honorato AMB, Varela H. Metallic single-atoms confined in carbon nanomaterials for the electrocatalysis of oxygen reduction, oxygen evolution, and hydrogen evolution reactions. Catal Sci Technol 2020;10:6420-48.
30. Fei H, Dong J, Arellano-Jiménez MJ, et al. Atomic cobalt on nitrogen-doped graphene for hydrogen generation. Nat Commun 2015;6:8668.
31. Qu Y, Chen B, Li Z, et al. Thermal emitting strategy to synthesize atomically dispersed Pt metal sites from bulk Pt metal. J Am Chem Soc 2019;141:4505-9.
32. Xue Y, Huang B, Yi Y, et al. Anchoring zero valence single atoms of nickel and iron on graphdiyne for hydrogen evolution. Nat Commun 2018;9:1460.
33. Lu B, Guo L, Wu F, et al. Ruthenium atomically dispersed in carbon outperforms platinum toward hydrogen evolution in alkaline media. Nat Commun 2019;10:631.
34. Zhang L, Si R, Liu H, et al. Atomic layer deposited Pt-Ru dual-metal dimers and identifying their active sites for hydrogen evolution reaction. Nat Commun 2019;10:4936.
35. Bai L, Hsu CS, Alexander DTL, Chen HM, Hu X. A cobalt-iron double-atom catalyst for the oxygen evolution reaction. J Am Chem Soc 2019;141:14190-9.
36. He T, Puente Santiago AR, Du A. Atomically embedded asymmetrical dual-metal dimers on N-doped graphene for ultra-efficient nitrogen reduction reaction. J Catal 2020;388:77-83.
37. Zheng X, Yao Y, Ye W, Gao P, Liu Y. Building up bimetallic active sites for electrocatalyzing hydrogen evolution reaction under acidic and alkaline conditions. Chem Eng J 2021;413:128027.
38. Zhou Y, Song E, Chen W, et al. Dual-metal interbonding as the chemical facilitator for single-atom dispersions. Adv Mater 2020;32:e2003484.
39. Zhao W, Luo C, Lin Y, et al. Pt-Ru dimer electrocatalyst with electron redistribution for hydrogen evolution reaction. ACS Catal 2022;12:5540-8.
40. Lu B, Liu Q, Chen S. Electrocatalysis of single-atom sites: impacts of atomic coordination. ACS Catal 2020;10:7584-618.
41. Perdew JP, Burke K, Ernzerhof M. Generalized gradient approximation made simple. Phys Rev Lett 1996;77:3865-8.
42. Kresse G, Furthmüller J. Efficient iterative schemes for ab initio total-energy calculations using a plane-wave basis set. Phys Rev B Condens Matter 1996;54:11169-86.
43. Grimme S, Antony J, Ehrlich S, Krieg H. A consistent and accurate ab initio parametrization of density functional dispersion correction (DFT-D) for the 94 elements H-Pu. J Chem Phys 2010;132:154104.
44. Zhang C, Qin S, Li B, Jin P. Dual-metal atom incorporated N-doped graphenes as oxygen evolution reaction electrocatalysts: high activities achieved by site synergies. J Mater Chem A 2022;10:8309-23.
45. Momma K, Izumi F. VESTA 3 for three-dimensional visualization of crystal, volumetric and morphology data. J Appl Cryst 2011;44:1272-6.
46. Yang TT, Wang A, House SD, Yang J, Lee JK, Saidi WA. Computationally guided design to accelerate discovery of doped β-Mo2C catalysts toward hydrogen evolution reaction. ACS Catal 2022;12:11791-800.
47. Nørskov JK, Rossmeisl J, Logadottir A, et al. Origin of the overpotential for oxygen reduction at a fuel-cell cathode. J Phys Chem B 2004;108:17886-92.
48. Hossain MD, Liu Z, Zhuang M, et al. Rational design of graphene-supported single atom catalysts for hydrogen evolution reaction. Adv Energy Mater 2019;9:1803689.
50. Lim J, Back S, Choi C, Jung Y. Ultralow overpotential of hydrogen evolution reaction using Fe-doped defective graphene: a density functional study. ChemCatChem 2018;10:4450-5.
51. Yang Y, Zhang H, Liang Z, et al. Role of local coordination in bimetallic sites for oxygen reduction: a theoretical analysis. J Energy Chem 2020;44:131-7.
52. Crabtree RH. Dihydrogen complexes: some structural and chemical studies. Acc Chem Res 1990;23:95-101.
53. Heinekey DM, Lledŏs A, Lluch JM. Elongated dihydrogen complexes: what remains of the H-H bond? Chem Soc Rev 2004;33:175-82.
54. Kubas GJ. Fundamentals of H2 binding and reactivity on transition metals underlying hydrogenase function and H2 production and storage. Chem Rev 2007;107:4152-205.
55. Kubas GJ. Molecular hydrogen complexes: coordination of a .sigma. bond to transition metals. Acc Chem Res 1988;21:120-8.
57. Alcaraz G, Grellier M, Sabo-Etienne S. Bis sigma-bond dihydrogen and borane ruthenium complexes: bonding nature, catalytic applications, and reversible hydrogen release. Acc Chem Res 2009;42:1640-9.
58. Di Liberto G, Cipriano LA, Pacchioni G. Role of dihydride and dihydrogen complexes in hydrogen evolution reaction on single-atom catalysts. J Am Chem Soc 2021;143:20431-41.
59. Di Liberto G, Cipriano LA, Pacchioni G. Single atom catalysts: what matters most, the active site or the surrounding? ChemCatChem 2022;14:e202200611.
Cite This Article
Export citation file: BibTeX | RIS
OAE Style
Zhang C, Qin S, Gao H, Jin P. High hydrogen evolution activities of dual-metal atoms incorporated N-doped graphenes achieved by coordination regulation. J Mater Inf 2024;4:1. http://dx.doi.org/10.20517/jmi.2023.34
AMA Style
Zhang C, Qin S, Gao H, Jin P. High hydrogen evolution activities of dual-metal atoms incorporated N-doped graphenes achieved by coordination regulation. Journal of Materials Informatics. 2024; 4(1): 1. http://dx.doi.org/10.20517/jmi.2023.34
Chicago/Turabian Style
Zhang, Cunjin, Shuaibo Qin, Hui Gao, Peng Jin. 2024. "High hydrogen evolution activities of dual-metal atoms incorporated N-doped graphenes achieved by coordination regulation" Journal of Materials Informatics. 4, no.1: 1. http://dx.doi.org/10.20517/jmi.2023.34
ACS Style
Zhang, C.; Qin S.; Gao H.; Jin P. High hydrogen evolution activities of dual-metal atoms incorporated N-doped graphenes achieved by coordination regulation. J. Mater. Inf. 2024, 4, 1. http://dx.doi.org/10.20517/jmi.2023.34
About This Article
Special Issue
Copyright
Data & Comments
Data
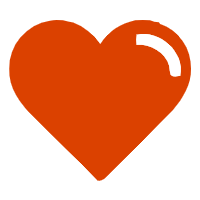

Comments
Comments must be written in English. Spam, offensive content, impersonation, and private information will not be permitted. If any comment is reported and identified as inappropriate content by OAE staff, the comment will be removed without notice. If you have any queries or need any help, please contact us at support@oaepublish.com.